Molecular Simulations have Boosted Knowledge of CRISPR/Cas9: A Review
DOI:
https://doi.org/10.13052/jsame2245-4551.7.003Keywords:
CRISPR/Cas9, genome editing, computer simulations, molecular dynamics, structure-function relationship.Abstract
Genome editing allows scientists to change an organism’s DNA. One promis-
ing genome editing protocol, already validated in living organisms, is based
on clustered regularly interspaced short palindromic repeats (CRISPR)/Cas
protein-nucleic acid complexes. When the CRISPR/Cas approach was first
demonstrated in 2012, its advantages with respect to previously available
techniques, such as zinc-finger nucleases (ZFNs) and transcription activator-
like effector nucleases (TALENs), immediately got attention and the method
has seen a surge of experimental and computational investigations since then.
However, the molecular mechanisms involved in target DNA recognition and
cleavage are still not completely resolved and need further attention. The large
size and complex nature of CRISPR/Cas9 complexes has been a challenge
for computational studies, but some seed results exist and are illuminating
on the cleavage activity. In this short review, we present recent progress
in studying CRISPR/Cas9 systems by molecular dynamics simulations with
coarse-grained and atomistic descriptions, including enhanced sampling.
Downloads
References
Baig, A.M. 2018. Human Genome-Edited Babies: First Responder
with Concerns Regarding Possible Neurological Deficits! ACS Chem.
Neurosci. 10: 8b00668.
Consortium, I.H.G.S. 2001. Initial sequencing and analysis of the human
genome. Nature. 409: 860–921.
Ishino, Y., H. Shinagawa, K. Makino, M. Amemura, and A. Nakata. 1987.
Nucleotide sequence of the iap gene, responsible for alkaline phosphatase
isozyme conversion in Escherichia coli, and identification of the gene
product. J. Bacteriol. 169: 5429–33.
Jinek, M., K. Chylinski, I. Fonfara, M. Hauer, J.A. Doudna, and
E. Charpentier. 2012. A programmable dual-RNA-guided DNA endonu-
clease in adaptive bacterial immunity. Science. 337: 816–21.
Molecular Simulations have Boosted Knowledge of CRISPR/Cas9: A Review 65
Cong, L., F.A. Ran, D. Cox, S. Lin, R. Barretto, N. Habib, P.D. Hsu, X.
Wu, W. Jiang, L.A. Marraffini, and F. Zhang. 2013. Multiplex genome
engineering using CRISPR/Cas systems. Science. 339: 819–23.
Jinek, M., F. Jiang, D.W. Taylor, S.H. Sternberg, E. Kaya, E. Ma,
C. Anders, M. Hauer, K. Zhou, S. Lin, M. Kaplan, A.T. Iavarone,
E. Charpentier, E. Nogales, and J.A. Doudna. 2014. Structures of
Cas9 Endonucleases Reveal RNA-Mediated Conformational Activation.
Science. 343: 1247997–1247997.
Nishimasu, H., F.A. Ran, P.D. Hsu, S. Konermann, S.I. Shehata,
N. Dohmae, R. Ishitani, F. Zhang, and O. Nureki. 2014. Crystal Structure
of Cas9 in Complex with Guide RNA and Target DNA. Cell. 156:
–949.
Karginov, F. V, and G.J. Hannon. 2010. The CRISPR system: small RNA-
guided defense in bacteria and archaea. Mol. Cell. 37: 7–19.
Rath, D., L. Amlinger, A. Rath, and M. Lundgren. 2015. The CRISPR-
Cas immune system: Biology, mechanisms and applications. Biochimie.
: 119–128.
Mohanraju, P., K.S. Makarova, B. Zetsche, F. Zhang, E. V. Koonin,
and J. van der Oost. 2016. Diverse evolutionary roots and mechanistic
variations of the CRISPR-Cas systems. Science. 353: aad5147.
Thurtle-Schmidt, D.M., and T.-W. Lo. 2018. Molecular biology at the cut-
ting edge: A review on CRISPR/CAS9 gene editing for undergraduates.
Biochem. Mol. Biol. Educ. 46: 195–205.
Makarova, K.S., D.H. Haft, R. Barrangou, S.J.J. Brouns, E. Charpentier,
P. Horvath, S. Moineau, F.J.M. Mojica, Y.I. Wolf, A.F. Yakunin, J. van
der Oost, and E. V Koonin. 2011. Evolution and classification of the
CRISPR-Cas systems. Nat. Rev. Microbiol. 9: 467–77.
Shmakov, S., A. Smargon, D. Scott, D. Cox, N. Pyzocha, W. Yan, O.O.
Abudayyeh, J.S. Gootenberg, K.S. Makarova, Y.I. Wolf, K. Severinov,
F. Zhang, and E. V. Koonin. 2017. Diversity and evolution of class 2
CRISPR–Cas systems. Nat. Rev. Microbiol. 15: 169–182.
Koonin, E. V, K.S. Makarova, and F. Zhang. 2017. Diversity, classifica-
tion and evolution of CRISPR-Cas systems. Curr. Opin. Microbiol. 37:
–78.
Swarts, D.C., and M. Jinek. 2018. Cas9 versus Cas12a/Cpf1: Structure-
function comparisons and implications for genome editing. Wiley
Interdiscip. Rev. RNA. 9: e1481.
Makarova, K.S., Y.I. Wolf, O.S. Alkhnbashi, F. Costa, S.A. Shah, S.J.
Saunders, R. Barrangou, S.J.J. Brouns, E. Charpentier, D.H. Haft, P.
A. Ray and R. Di Felice
Horvath, S. Moineau, F.J.M. Mojica, R.M. Terns, M.P. Terns, M.F.
White, A.F. Yakunin, R.A. Garrett, J. van der Oost, R. Backofen, and E.V.
Koonin. 2015. An updated evolutionary classification of CRISPR–Cas
systems. Nat. Rev. Microbiol. 13: 722–736.
Makarova, K.S., Y.I. Wolf, and E. V. Koonin. 2018. Classification and
Nomenclature of CRISPR-Cas Systems: Where from Here? Cris. J. 1:
–336.
Jiang, F., and J.A. Doudna. 2017. CRISPR–Cas9 Structures and Mecha-
nisms. Annu. Rev. Biophys. 46: 505–529.
Sternberg, S.H., S. Redding, M. Jinek, E.C. Greene, and J.A. Doudna.
DNA interrogation by the CRISPR RNA-guided endonuclease
Cas9. Nature. 507: 62–67.
Gong, S., H.H. Yu, K.A. Johnson, and D.W. Taylor. 2018. DNA Unwind-
ing Is the Primary Determinant of CRISPR-Cas9 Activity. Cell Rep. 22:
–371.
Deltcheva, E., K. Chylinski, C.M. Sharma, K. Gonzales, Y. Chao, Z.A.
Pirzada, M.R. Eckert, J. Vogel, and E. Charpentier. 2011. CRISPR RNA
maturation by trans-encoded small RNA and host factor RNase III.
Nature. 471: 602–607.
Jinek, M., K. Chylinski, I. Fonfara, M. Hauer, J.A. Doudna,
and E. Charpentier. 2012. A Programmable Dual-RNA–Guided DNA
Endonuclease in Adaptive Bacterial Immunity. Science. 337: 816–821.
Hille, F., and E. Charpentier. 2016. CRISPR-Cas: biology, mechanisms
and relevance. Philos. Trans. R. Soc. Lond. B. Biol. Sci. 371.
Rueda, F.O., M. Bista, M.D. Newton, A.U. Goeppert, M.E. Cuomo,
E. Gordon, F. Kr ̈oner, J.A. Read, J.D. Wrigley, D. Rueda, and B.J.M.
Taylor. 2017. Mapping the sugar dependency for rational generation of a
DNA-RNA hybrid-guided Cas9 endonuclease. Nat. Commun. 8: 1610.
CRISPR/Cas9 & Targeted Genome Editing: New Era in Molecular
Biology. https://www.neb.com/tools-and-resources/feature-articles/cris
pr-cas9-and-targeted-genome-editing-a-new-era-in-molecular-biology
Lee, J., J.-H. Chung, H.M. Kim, D.-W. Kim, and H. Kim. 2016. Designed
nucleases for targeted genome editing. Plant Biotechnol. J. 14: 448–462.
Anders, C., O. Niewoehner, A. Duerst, and M. Jinek. 2014. Struc-
tural basis of PAM-dependent target DNA recognition by the Cas9
endonuclease. Nature. 513: 569–573.
Jiang, F., D.W. Taylor, J.S. Chen, J.E. Kornfeld, K. Zhou, A.J. Thompson,
E. Nogales, and J.A. Doudna. 2016. Structures of a CRISPR-Cas9 R-loop
complex primed for DNA cleavage. Science. 351: 867–871.
Molecular Simulations have Boosted Knowledge of CRISPR/Cas9: A Review 67
Slaymaker, I.M., L. Gao, B. Zetsche, D.A. Scott, W.X. Yan, and F. Zhang.
Rationally engineered Cas9 nucleases with improved specificity.
Science. 351: 84–88.
Palermo, G., Y. Miao, R.C. Walker, M. Jinek, and J.A. McCammon. 2017.
CRISPR-Cas9 conformational activation as elucidated from enhanced
molecular simulations. Proc. Natl. Acad. Sci. U. S. A. 114: 7260–7265.
Tangprasertchai, N.S., R. Di Felice, X. Zhang, I.M. Slaymaker, C.
Vazquez Reyes, W. Jiang, R. Rohs, and P.Z. Qin. 2017. CRISPR–Cas9
Mediated DNA Unwinding Detected Using Site-Directed Spin Labeling.
ACS Chem. Biol. 12: 1489–1493.
Humphrey, W., A. Dalke, and K. Schulten. 1996. VMD: visual molecular
dynamics. J. Mol. Graph. 14: 33–8, 27–8.
Jinek, M., A. East, A. Cheng, S. Lin, E. Ma, and J. Doudna. 2013. RNA-
programmed genome editing in human cells. 2: 471.
Mali, P., L. Yang, K.M. Esvelt, J. Aach, M. Guell, J.E. DiCarlo,
J.E. Norville, and G.M. Church. 2013. RNA-guided human genome
engineering via Cas9. Science. 339: 823-826
Zuo, Z., and J. Liu. 2016. Cas9-catalyzed DNA Cleavage Generates
Staggered Ends: Evidence from Molecular Dynamics Simulations. Sci.
Rep. 6: 37584.
Yang, W. 2011. Nucleases: diversity of structure, function and mecha-
nism. Q. Rev. Biophys. 44: 1–93.
Yang, W. 2008. An equivalent metal ion in one- and two-metal-ion
catalysis. Nat. Struct. Mol. Biol. 15: 1228–1231.
Yang, W., J.Y. Lee, and M. Nowotny. 2006. Making and Breaking Nucleic
Acids: Two-Mg2+-Ion Catalysis and Substrate Specificity. Mol. Cell. 15:
–123.
Li, P., and K.M. Merz. 2017. Metal Ion Modeling Using Classical
Mechanics. Chem. Rev. 117: 1564–1686.
Palermo, G. 2019. Structure and Dynamics of the CRISPR–Cas9
Catalytic Complex. J. Chem. Inf. Model. 59: 2394–2406.
Estarellas, C., M. Otyepka, J. Koˇca, P. Ban ́aˇs, M. Krepl, and
J. ˇSponer. 2015. Molecular dynamic simulations of protein/RNA com-
plexes: CRISPR/Csy4 endoribonuclease. Biochim. Biophys. Acta – Gen.
Subj. 1850: 1072–1090.
Shaw, D.E., J.P. Grossman, J.A. Bank, B. Batson, J.A. Butts, J.C. Chao,
M.M. Deneroff, R.O. Dror, A. Even, C.H. Fenton, A. Forte, J. Gagliardo,
G. Gill, B. Greskamp, C.R. Ho, D.J. Ierardi, L. Iserovich, J.S. Kuskin,
R.H. Larson, T. Layman, L.-S. Lee, A.K. Lerer, C. Li, D. Killebrew,
A. Ray and R. Di Felice
K.M. Mackenzie, S.Y.-H. Mok, M.A. Moraes, R. Mueller, L.J. Nociolo,
J.L. Peticolas, T. Quan, D. Ramot, J.K. Salmon, D.P. Scarpazza, U. Ben
Schafer, N. Siddique, C.W. Snyder, J. Spengler, P.T.P. Tang, M. Theobald,
H. Toma, B. Towles, B. Vitale, S.C. Wang, and C. Young. 2014. Anton 2:
Raising the Bar for Performance and Programmability in a Special-
Purpose Molecular Dynamics Supercomputer. In: SC14: International
Conference for High Performance Computing, Networking, Storage and
Analysis. IEEE. pp. 41–53.
Zheng, W. 2017. Probing the structural dynamics of the CRISPR-
Cas9 RNA-guided DNA-cleavage system by coarse-grained modeling.
Proteins Struct. Funct. Bioinforma. 85: 342–353.
Zhu, X., R. Clarke, A.K. Puppala, S. Chittori, A. Merk, B.J. Merrill,
M. Simonovic, and S. Subramaniam. 2019. Cryo-EM structures reveal
coordinated domain motions that govern DNA cleavage by Cas9. Nat.
Struct. Mol. Biol. 10.2210/PDB6O0X/PDB.
Guo, T.W., A. Bartesaghi, H. Yang, L.A. Earl, D.J. Patel, et al., 2017.
Cryo-EM Structures Reveal Mechanism and Inhibition of DNATargeting
by a CRISPR-Cas Surveillance Complex Data Resources 6B44 6B45
B46 6B47 6B48. Cell. 171: 414–426.
Dorsey, B.W., L. Huang, and A. Mondrag ́on. 2019. Structural organiza-
tion of a Type III-A CRISPR effector subcomplex determined by X-ray
crystallography and cryo-EM. Nucleic Acids Res. 47: 3765–3783.
Huo, Y., T. Li, N. Wang, Q. Dong, X. Wang, and T. Jiang. 2018. Cryo-
EM structure of Type III-A CRISPR effector complex. Cell Res. 28:
–1197.
Palermo, G., Y. Miao, R.C. Walker, M. Jinek, and J.A. McCammon. 2016.
Striking Plasticity of CRISPR-Cas9 and Key Role of Non-target DNA,
as Revealed by Molecular Simulations. ACS Cent. Sci. 2: 756–763.
Jiang, F., K. Zhou, L. Ma, S. Gressel, and J.A. Doudna. 2015. STRUC-
TURAL BIOLOGY. A Cas9-guide RNA complex preorganized for target
DNA recognition. Science. 348: 1477–81.
Sternberg, S.H., B. LaFrance, M. Kaplan, and J.A. Doudna. 2015. Con-
formational control of DNA target cleavage by CRISPR–Cas9. Nature.
: 110–113.
Dagdas, Y.S., J.S. Chen, S.H. Sternberg, J.A. Doudna, and A. Yildiz.
A conformational checkpoint between DNA binding and cleavage
by CRISPR-Cas9. Sci. Adv. 3: eaao0027.
Molecular Simulations have Boosted Knowledge of CRISPR/Cas9: A Review 69
Palermo, G., C.G. Ricci, A. Fernando, R. Basak, M. Jinek, I. Rivalta,
V.S. Batista, and J.A. McCammon. 2017. Protospacer Adjacent Motif-
Induced Allostery Activates CRISPR-Cas9. J. Am. Chem. Soc. 139:
–16031.
East, K.W., J.C. Newton, U.N. Morzan, A. Acharya, E. Skeens,
G. Jogl, V.S. Batista, G. Palermo, and G.P. Lisi. 2019. Allosteric Motions
of the CRISPR-Cas9 HNH Nuclease Probed by NMR and Molecular
Dynamics. bioRxiv.: 660613.
Zuo, Z., and J. Liu. 2017. Structure and Dynamics of Cas9 HNH Domain
Catalytic State. Sci. Rep. 7: 17271.
Yoon, H., L.N. Zhao, and A. Warshel. 2019. Exploring the Catalytic
Mechanism of Cas9 Using Information Inferred from Endonuclease VII.
ACS Catal. 9: 1329–1336.
Steitz, T.A., and J.A. Steitz. 1993. A general two-metal-ion mechanism
for catalytic RNA. Proc. Natl. Acad. Sci. U. S. A. 90: 6498–502.
Palermo, G., J.S. Chen, C.G. Ricci, I. Rivalta, M. Jinek, V.S. Batista, J.A.
Doudna, and J.A. McCammon. 2018. Key role of the REC lobe during
CRISPR–Cas9 activation by ‘sensing’, ‘regulating’, and ‘locking’ the
catalytic HNH domain. Q. Rev. Biophys. 51: e9.
Kleinstiver, B.P., V. Pattanayak, M.S. Prew, S.Q. Tsai, N.T. Nguyen, Z.
Zheng, and J.K. Joung. 2016. High-fidelity CRISPR–Cas9 nucleases with
no detectable genome-wide off-target effects. Nature. 529: 490–495.
Fu, Y., J.A. Foden, C. Khayter, M.L. Maeder, D. Reyon, J.K. Joung, and
J.D. Sander. 2013. High-frequency off-target mutagenesis induced by
CRISPR-Cas nucleases in human cells. Nat. Biotechnol. 31: 822–6.
Ricci, C.G., J.S. Chen, Y. Miao, M. Jinek, J.A. Doudna, J.A.
McCammon, and G. Palermo. 2019. Deciphering Off-Target Effects in
CRISPR-Cas9 through Accelerated Molecular Dynamics. ACS Cent.
Sci.: acscentsci.9b00020.
Zheng, L., J. Shi, and Y. Mu. 2018. Dynamics changes of CRISPR-Cas9
systems induced by high fidelity mutations. Phys. Chem. Chem. Phys.
: 27439–27448.
Qin, P.Z., I.S. Haworth, Q. Cai, A.K. Kusnetzow, G.P.G. Grant, E.A.
Price, G.Z. Sowa, A. Popova, B. Herreros, and H. He. 2007. Measuring
nanometer distances in nucleic acids using a sequence-independent
nitroxide probe. Nat. Protoc. 2: 2354–2365.
Hsu, P.D., D.A. Scott, J.A. Weinstein, F.A. Ran, S. Konermann,
V. Agarwala, Y. Li, E.J. Fine, X. Wu, O. Shalem, T.J. Cradick, L.A.
A. Ray and R. Di Felice
Marraffini, G. Bao, and F. Zhang. 2013. DNA targeting specificity of
RNA-guided Cas9 nucleases. Nat. Biotechnol. 31: 827–832.
Ray, A., and R. Di Felice. Unpublished.
Sundaresan, R., H.P. Parameshwaran, S.D. Yogesha, M.W. Keilbarth, and
R. Rajan. 2017. RNA-Independent DNA Cleavage Activities of Cas9 and
Cas12a. Cell Rep. 21: 3728–3739
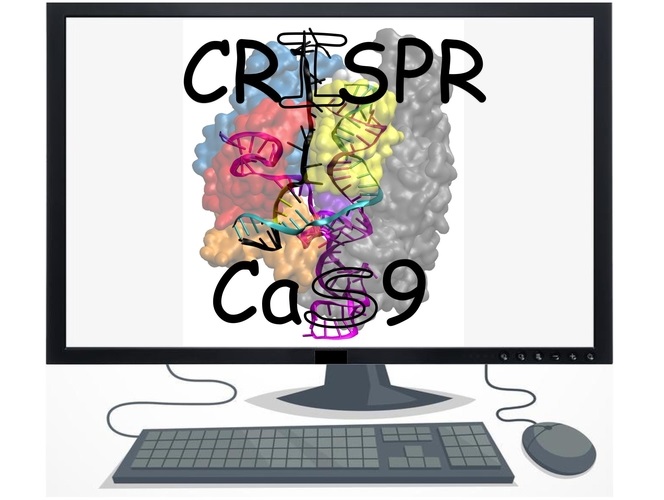